How GPS Works: The Technology That Maps the World
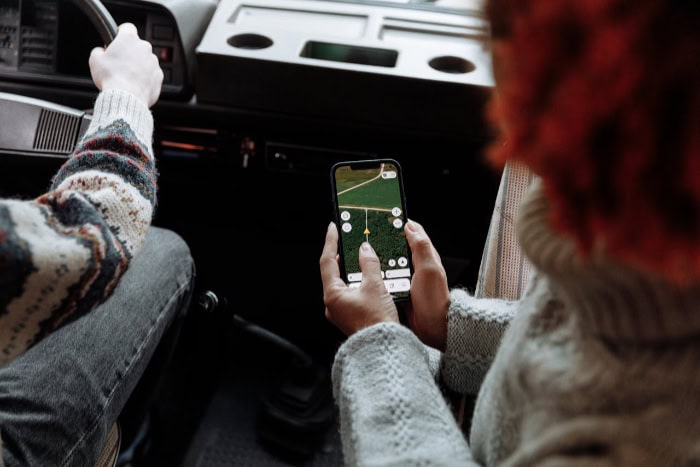
Picture yourself lost in an unfamiliar city, trying to navigate to your destination without a map. Just a few decades ago, this scenario would have been a common and frustrating experience.
However, thanks to the advent of the Global Positioning System (GPS), finding your way has become as simple as glancing at your smartphone or in-car navigation device. GPS technology has not only transformed personal navigation but has also become a critical tool in fields ranging from aviation to agriculture.
Fundamental Components
The Global Positioning System (GPS) relies on two primary components to function effectively: the Space Segment and the Ground Control Segment. These elements work in tandem to provide accurate positioning and timing information to users worldwide.
Space Segment
The Space Segment forms the backbone of the GPS network, consisting of a constellation of satellites orbiting the Earth. This segment is designed to ensure global coverage and continuous availability of GPS signals.
At the core of the Space Segment is a network of at least 24 satellites, though the current constellation typically includes more than 30 operational satellites.
These satellites orbit at an altitude of approximately 20,200 kilometers (12,550 miles) above the Earth’s surface in medium Earth orbit.
They are arranged in six orbital planes, each inclined at 55 degrees to the equator, ensuring that at least four satellites are visible from any point on Earth at any given time.
Each GPS satellite is equipped with highly precise atomic clocks, which are essential for accurate timekeeping. These clocks are typically cesium or rubidium-based and can maintain time accuracy to within a few nanoseconds per day.
This extreme precision is crucial for calculating accurate positions on Earth.
The satellites also carry sophisticated signal transmission equipment. This hardware generates and broadcasts the GPS signals that receivers on Earth use to determine their position.
The signals contain information about the satellite’s location, the time the signal was sent, and the health status of the satellite.
To power these complex systems, GPS satellites rely on solar energy. Large solar panels extend from the body of each satellite, converting sunlight into electricity to run all onboard systems.
In addition to solar power, the satellites are equipped with backup batteries to ensure continuous operation during periods when they pass through the Earth’s shadow.
The Space Segment also incorporates redundancy and backup systems to maintain the reliability of the GPS network.
If a satellite fails or needs to be taken offline for maintenance, the remaining satellites can adjust their positions slightly to compensate and maintain global coverage.
Ground Control
The Ground Control Segment, also known as the Control Segment, plays a vital role in managing and maintaining the GPS network. This segment consists of a global network of monitoring stations, a master control station, and several ground antennas.
The network of monitoring stations is spread across the globe, with locations carefully chosen to provide comprehensive coverage of the satellite constellation.
These stations continuously track GPS satellites as they pass overhead, collecting data on their signals, orbits, and overall health.
The data gathered by these monitoring stations is crucial for maintaining the accuracy and reliability of the GPS system.
At the heart of the Ground Control Segment is the master control station, located at Schriever Air Force Base in Colorado, USA. This facility serves as the nerve center for the entire GPS network.
It processes the data collected by the monitoring stations, computes precise orbital data (known as ephemeris) for each satellite, and generates the navigation messages that satellites broadcast to users.
The master control station is responsible for several critical functions. It monitors the health and status of all GPS satellites, ensuring they are functioning correctly and providing accurate information.
If any anomalies or issues are detected, the station can take corrective action, such as adjusting a satellite’s orbit or switching to backup systems.
Regular satellite maintenance and updates are another crucial aspect of the Ground Control Segment’s responsibilities.
This includes uploading new navigation data to the satellites, adjusting their orbits when necessary, and performing software updates to improve performance or address potential vulnerabilities.
The Ground Control Segment also includes several ground antennas located around the world. These antennas are used to communicate with the GPS satellites, transmitting updated navigation data and commands from the master control station.
Signal Transmission Process
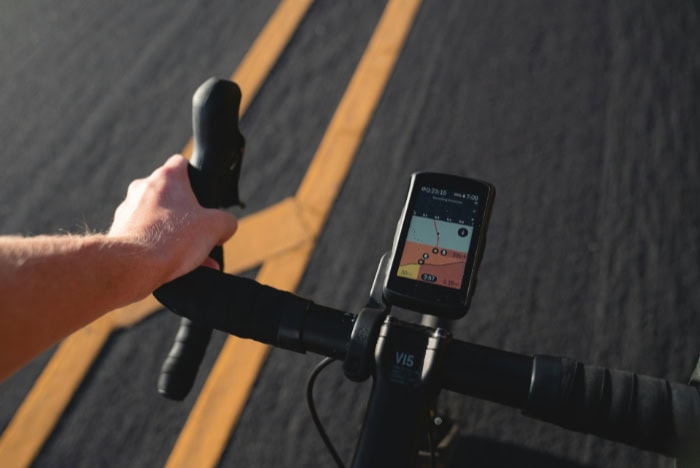
The signal transmission process is the backbone of the Global Positioning System (GPS), enabling communication between satellites and receivers on Earth. This process involves the continuous broadcasting of radio signals from the satellites and the reception and processing of these signals by GPS receivers.
Satellite Broadcasting
GPS satellites are constantly transmitting radio signals that carry crucial information for determining positions on Earth. Each satellite broadcasts on several frequencies, with the primary frequency being L1 at 1575.42 MHz.
This frequency is used for the Coarse/Acquisition (C/A) code, which is accessible to civilian users. The L2 frequency, at 1227.60 MHz, is used for the Precise (P) code and is primarily reserved for military and authorized users.
The radio signals transmitted by GPS satellites contain time-stamped messages that include information about the satellite’s position, the time the signal was sent, and the health status of the satellite.
These messages are generated using a precise atomic clock onboard each satellite, ensuring that the time information is highly accurate.
The signals are broadcast using a technique called Code Division Multiple Access (CDMA), which allows multiple satellites to transmit on the same frequency without interfering with each other.
Each satellite is assigned a unique pseudorandom noise (PRN) code that identifies its signals. This code is used to modulate the carrier frequency, creating a spread-spectrum signal that is resistant to interference and jamming.
The GPS signals have specific frequency and wavelength characteristics that enable them to travel through the Earth’s atmosphere and reach receivers on the ground. The L1 and L2 frequencies are chosen because they are less susceptible to atmospheric disturbances, such as ionospheric delay, which can affect the accuracy of position calculations.
Signal Reception
GPS receivers are designed to pick up the radio signals transmitted by the satellites and use the information contained in these signals to calculate the receiver’s position on Earth. The receiver’s antenna is tuned to the specific frequencies used by GPS satellites, allowing it to capture the incoming signals.
Once the signals are received, the GPS receiver begins processing and decoding the information. The receiver generates a replica of the PRN code for each satellite it is tracking and uses this code to correlate with the incoming signals.
By measuring the time delay between the transmission of the signal from the satellite and its arrival at the receiver, the distance between the satellite and the receiver can be calculated. This distance is known as the pseudorange.
To accurately determine its position, a GPS receiver needs to acquire signals from at least four satellites. The receiver uses the pseudoranges from these satellites to calculate its position in three dimensions (latitude, longitude, and altitude) through a process called trilateration.
The fourth satellite is necessary to correct for any timing errors between the receiver’s clock and the atomic clocks onboard the satellites.
GPS receivers are designed to handle multiple satellite signals simultaneously. Modern receivers can track up to 20 or more satellites at once, improving the accuracy and reliability of position calculations.
The receiver continuously updates its position as it receives new signals, allowing for real-time tracking and navigation.
Advanced GPS receivers also incorporate various signal processing techniques to improve the accuracy and reliability of position calculations.
These techniques include multipath mitigation, which reduces the impact of signals that have bounced off objects before reaching the receiver, and differential GPS, which uses corrections from a known reference station to improve accuracy.
The signal transmission process, from the broadcasting of radio signals by satellites to the reception and processing of these signals by GPS receivers, forms the foundation of GPS technology.
Position Calculation
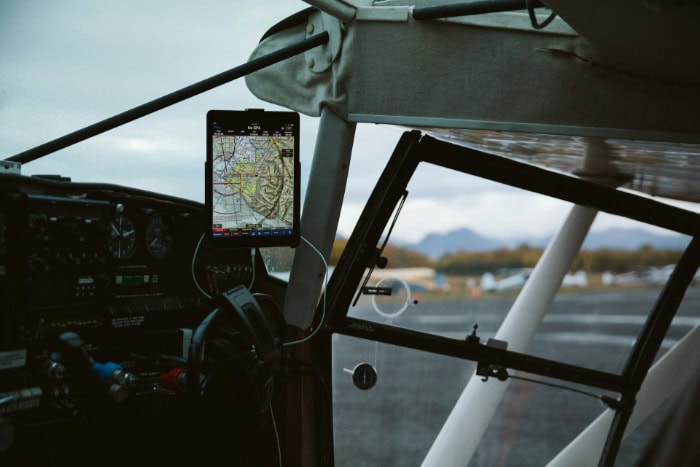
The heart of GPS technology lies in its ability to accurately calculate a receiver’s position on Earth. This process involves complex mathematical calculations based on the signals received from multiple satellites.
Two critical components of position calculation are the trilateration method and timing synchronization. These elements work together to provide the precise location information that GPS users rely on every day.
Trilateration Method
Trilateration is the fundamental technique used by GPS receivers to determine their position on Earth. This method involves measuring the distances between the receiver and multiple satellites to pinpoint the receiver’s exact location.
The process is similar to finding a point on a map by knowing its distance from several known landmarks.
In GPS, each satellite acts as a reference point in space with a known position. The receiver measures its distance from at least four satellites by calculating the time it takes for the signal to travel from the satellite to the receiver.
This distance measurement creates a sphere around each satellite, with the radius equal to the distance between the satellite and the receiver.
The intersection of these spheres narrows down the possible locations of the receiver. With signals from three satellites, the intersection of their spheres creates two points in space where the receiver could be located.
However, one of these points is typically far from Earth’s surface and can be discarded. The fourth satellite measurement is crucial for confirming the receiver’s position and correcting for timing errors.
The minimum requirement of four satellites is essential for accurate three-dimensional positioning. Three satellites provide latitude and longitude information, while the fourth satellite adds altitude data and resolves timing discrepancies.
In practice, GPS receivers often track more than four satellites to improve accuracy and provide redundancy in case of signal loss from one or more satellites.
The trilateration process is performed continuously by the GPS receiver, updating the position as new signals are received.
This constant calculation allows for real-time tracking and navigation, enabling applications such as turn-by-turn directions in vehicle navigation systems.
Timing Synchronization
Precise timing is crucial for accurate GPS positioning. The system relies on extremely accurate measurements of the time it takes for signals to travel from satellites to receivers. Even a tiny error in timing can result in significant positioning errors.
This is where atomic clocks and sophisticated timing synchronization techniques come into play.
Each GPS satellite carries multiple atomic clocks that provide incredibly precise timekeeping. These clocks are accurate to within a few nanoseconds per day, which is essential for measuring the travel time of radio signals that move at the speed of light.
The atomic clocks ensure that all satellites in the GPS constellation are synchronized to the same time reference.
However, GPS receivers typically use less accurate quartz clocks, which can lead to timing discrepancies. To address this issue, GPS employs time correction algorithms.
The receiver uses the signals from four or more satellites to solve for four unknowns: latitude, longitude, altitude, and time. By including time as an unknown in the calculation, the receiver can correct for any offset between its internal clock and the GPS system time.
This process of timing synchronization is performed with each position calculation, ensuring that the receiver’s clock remains closely aligned with GPS system time.
The continuous correction of timing errors is what allows relatively inexpensive GPS receivers to achieve high levels of accuracy without the need for atomic clocks in every device.
Error compensation techniques are also employed to further improve the accuracy of GPS positioning.
These techniques account for various sources of error, such as atmospheric delays, multipath effects (signals reflecting off buildings or other objects), and satellite orbit inaccuracies.
Advanced GPS receivers use sophisticated algorithms to model and compensate for these errors, resulting in more precise position calculations.
One such technique is differential GPS (DGPS), which uses a network of fixed, ground-based reference stations to broadcast the difference between the positions indicated by the GPS satellite systems and the known fixed positions.
These corrections can be applied to nearby GPS receivers, significantly improving their accuracy.
Another important error compensation technique is the use of augmentation systems, such as the Wide Area Augmentation System (WAAS) in North America. These systems provide additional satellite signals and error corrections that enhance the accuracy and reliability of GPS positioning.
The combination of trilateration and precise timing synchronization, along with sophisticated error compensation techniques, allows GPS to provide accurate position information to users around the globe.
Accuracy Factors
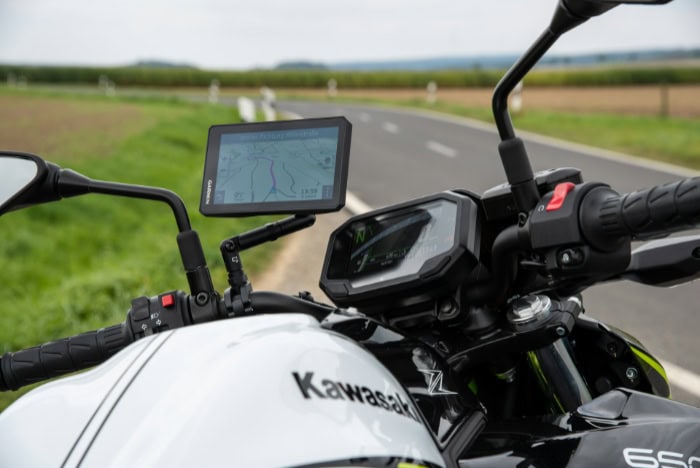
While GPS technology has reshaped navigation and positioning, several factors can influence the accuracy of the system. These factors can be broadly categorized into environmental influences and system limitations.
Environmental Influences
The environment in which GPS signals travel and are received can have a significant impact on the accuracy of position calculations. Three primary environmental factors affect GPS accuracy: atmospheric effects, physical obstacles, and signal reflection and multipath.
Atmospheric effects, particularly ionospheric and tropospheric delays, can cause GPS signals to slow down or speed up as they pass through the Earth’s atmosphere.
The ionosphere, a layer of the upper atmosphere containing charged particles, can refract GPS signals, causing them to take longer to reach the receiver.
The troposphere, the lower part of the atmosphere, can also delay signals due to variations in temperature, pressure, and humidity.
These atmospheric delays can introduce errors in the calculated distances between satellites and receivers, affecting the accuracy of position estimates.
Physical obstacles, such as buildings, mountains, and dense foliage, can block or attenuate GPS signals, making it difficult for receivers to acquire and track satellites.
In urban environments, tall buildings can create “urban canyons” that limit satellite visibility and reduce the number of signals available for position calculation.
Similarly, in heavily forested areas or deep valleys, GPS signals may be weakened or blocked entirely, compromising positioning accuracy.
Signal reflection and multipath occur when GPS signals bounce off surfaces like buildings, water, or the ground before reaching the receiver. These reflected signals can interfere with the direct signals from satellites, leading to inaccurate distance measurements.
Multipath effects are particularly problematic in urban areas with many reflective surfaces, such as glass and metal structures.
Advanced GPS receivers use various techniques, such as antenna design and signal processing algorithms, to mitigate the impact of multipath and improve accuracy in challenging environments.
System Limitations
In addition to environmental factors, GPS accuracy is also subject to inherent limitations of the system itself. Three notable system limitations are geometric dilution of precision, signal availability constraints, and hardware capabilities.
Geometric dilution of precision (GDOP) refers to the impact of satellite geometry on position accuracy. The arrangement of satellites in the sky relative to the receiver can affect the precision of position calculations.
When satellites are clustered together or in a line, the geometry is said to be weak, resulting in higher GDOP values and lower accuracy. Conversely, when satellites are spread out across the sky, the geometry is strong, leading to lower GDOP values and better accuracy.
GPS receivers typically aim to select satellites with the best geometry to minimize GDOP and optimize position accuracy.
Signal availability constraints can also limit GPS accuracy. The GPS system is designed to provide global coverage, but the number of satellites visible from any given location can vary.
In order to calculate a position, a receiver needs signals from at least four satellites. However, in some situations, such as in deep urban canyons or under heavy tree cover, the number of visible satellites may drop below four, making it impossible to determine a position.
In these cases, GPS receivers may need to rely on other positioning technologies, such as inertial navigation systems or Wi-Fi positioning, to supplement GPS and maintain a level of accuracy.
Hardware capabilities of GPS receivers can also impact accuracy. The quality of the receiver’s antenna, clock, and signal processing components can affect its ability to acquire and track satellite signals, as well as the precision of its position calculations.
High-end GPS receivers often feature more advanced hardware, such as multi-frequency antennas and more precise clocks, which can improve accuracy and performance in challenging environments. However, these advanced features come at a higher cost, and not all applications require the same level of hardware sophistication.
It’s important to note that GPS accuracy can be improved through various techniques, such as differential GPS (DGPS), real-time kinematic (RTK) positioning, and the use of augmentation systems like the Wide Area Augmentation System (WAAS) or the European Geostationary Navigation Overlay Service (EGNOS).
These techniques involve the use of reference stations or additional satellite signals to provide corrections and enhancements to the standard GPS signals, resulting in higher accuracy and reliability.
Real-World Applications
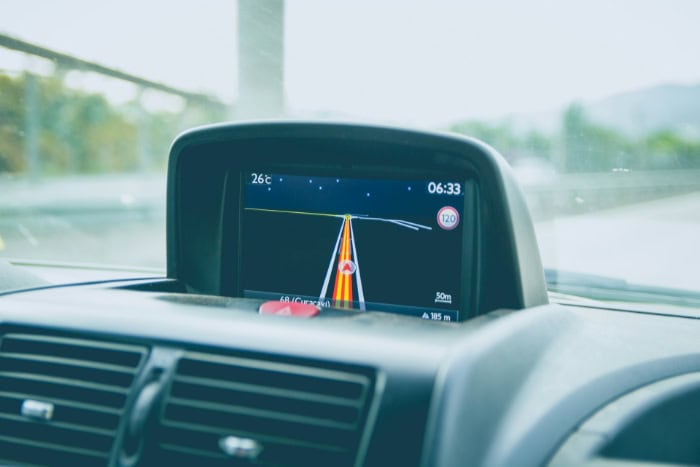
The Global Positioning System (GPS) has found its way into numerous aspects of our daily lives and professional fields. From helping us navigate unfamiliar streets to supporting critical military operations, GPS technology has become an indispensable tool in modern society.
Navigation Systems
GPS-based navigation systems have transformed the way we travel and move around in our environment. These systems provide accurate, real-time positioning information that enables users to determine their location and find the best routes to their destinations.
Vehicle navigation is perhaps the most widely recognized application of GPS technology. In-car GPS systems and smartphone apps have made paper maps largely obsolete for many drivers.
These systems offer turn-by-turn directions, estimated arrival times, and real-time traffic information.
Advanced features include suggesting alternative routes to avoid congestion, locating nearby points of interest such as gas stations or restaurants, and even providing parking assistance in some urban areas.
The impact of GPS on maritime and aviation navigation has been equally significant. In maritime applications, GPS helps ships navigate safely through crowded waterways, avoid hazards, and maintain efficient routes across vast oceans.
Modern vessels use GPS in conjunction with electronic charts and other navigational aids to enhance safety and improve operational efficiency. For aviation, GPS has become a critical component of navigation and air traffic control systems.
It enables pilots to fly more direct routes, reducing fuel consumption and flight times. GPS also enhances safety by providing precise positioning information during takeoffs, landings, and in low-visibility conditions.
Pedestrian guidance has also benefited greatly from GPS technology. Smartphone apps and dedicated handheld GPS devices help hikers, tourists, and urban explorers find their way in unfamiliar environments.
These systems can provide walking directions, locate nearby public transportation options, and even offer augmented reality features that overlay directional information on real-world views through the device’s camera.
Specialized Uses
Beyond everyday navigation, GPS technology plays a crucial role in various specialized fields, including military operations, scientific research, and emergency services.
In military operations, GPS has become an essential tool for strategic planning, troop movement, and weapon guidance.
Military forces use GPS for precise positioning of personnel and equipment, coordinating complex operations across large areas, and navigating in unfamiliar or hostile territories.
GPS-guided munitions have significantly improved the accuracy of weapon systems, reducing collateral damage and increasing operational effectiveness. Additionally, GPS timing capabilities are crucial for synchronizing military communications and encryption systems.
Scientific research benefits from GPS in numerous ways. Geologists use GPS to measure tectonic plate movements and monitor volcanic activity with millimeter-level precision.
Wildlife biologists track animal migrations and study habitat use by fitting animals with GPS collars. Climate scientists employ GPS to measure sea level changes and ice sheet movements, contributing to our understanding of global climate patterns.
In agriculture, GPS-guided precision farming techniques help optimize crop yields and reduce resource use by enabling targeted application of water, fertilizers, and pesticides.
Emergency services rely heavily on GPS technology to respond quickly and effectively to crises. 911 dispatch systems use GPS to locate callers and direct first responders to emergency scenes.
Search and rescue operations utilize GPS to coordinate teams and locate missing persons in remote areas. During natural disasters, GPS helps emergency managers track the movement of resources, assess damage, and plan recovery efforts.
Ambulance services use GPS navigation to find the fastest routes to hospitals, potentially saving lives by reducing response times.
The applications of GPS technology in these specialized fields continue to expand as the system’s accuracy and reliability improve.
Innovations such as high-precision GPS and integration with other sensors and data sources are opening up new possibilities for using GPS in increasingly sophisticated ways.
Conclusion
Global Positioning System technology has become an indispensable part of modern life, seamlessly integrating into our daily routines and professional endeavors.
From its intricate network of satellites orbiting Earth to the complex calculations performed by receivers, GPS exemplifies human ingenuity in solving complex problems.
The system’s ability to provide accurate location and timing information has transformed navigation, scientific research, and emergency services.
Despite challenges posed by environmental factors and system limitations, ongoing advancements continue to enhance GPS accuracy and reliability.
As we look to the future, GPS will undoubtedly play an even more significant role in shaping our world, driving innovation across various sectors and opening new possibilities for location-based technologies. The story of GPS serves as a powerful reminder of how scientific progress can profoundly impact society, connecting us to our surroundings with unprecedented precision.